Abstract
The steady-state heat transfer in laminar flow of liquid egg yolk - an important pseudoplastic fluid food - in circular and concentric annular ducts was experimentally investigated. The average convection heat transfer coefficients, determined by measuring temperatures before and after heating sections with constant temperatures at the tube wall, were used to obtain simple new empirical expressions to estimate the Nusselt numbers for fully established flows at the thermal entrance of the considered geometries. The comparisons with existing correlations for Newtonian and non-Newtonian fluids resulted in excellent agreement. The main contribution of this work is to supply practical and easily applicable correlations, which are, especially for the case of annulus, rather scarce and extensively required in the design of heat transfer operations dealing with similar shear-thinning products. In addition, the experimental results may support existing theoretical analyses.
Egg yolk; Heat transfer; Laminar flow; Nusselt number
FLUID DYNAMICS; HEAT AND MASS TRANSFER; AND OTHER TOPICS
Forced convection to laminar flow of liquid egg yolk in circular and annular ducts
M. BernardiI; V. Silveira Jr.I; V. R. N. TelisII; A. L. GabasIII; J. Telis-RomeroII,* * To whom correspondence should be addressed
IUniversidade Estadual de Campinas, UNICAMP, Departamento de Engenharia de Alimentos, 13083-862, Campinas - SP, Brasil
IIUniversidade Estadual Paulista, UNESP, Departamento de Engenharia e Tecnologia de Alimentos, 15054-000, São José do Rio Preto - SP, Brasil. E-mail: javier@ibilce.unesp.br
IIIUniversidade de São Paulo, USP, Departamento de Engenharia de Alimentos, FZEA, 13635-900, Pirassununga - SP, Brasil
ABSTRACT
The steady-state heat transfer in laminar flow of liquid egg yolk - an important pseudoplastic fluid food - in circular and concentric annular ducts was experimentally investigated. The average convection heat transfer coefficients, determined by measuring temperatures before and after heating sections with constant temperatures at the tube wall, were used to obtain simple new empirical expressions to estimate the Nusselt numbers for fully established flows at the thermal entrance of the considered geometries. The comparisons with existing correlations for Newtonian and non-Newtonian fluids resulted in excellent agreement. The main contribution of this work is to supply practical and easily applicable correlations, which are, especially for the case of annulus, rather scarce and extensively required in the design of heat transfer operations dealing with similar shear-thinning products. In addition, the experimental results may support existing theoretical analyses.
Keywords: Egg yolk; Heat transfer; Laminar flow; Nusselt number.
INTRODUCTION
The major technical applications of laminar flow forced convection heat transfer in closed conduits are in the analysis and design of heat exchangers. The convective heat transfer coefficients for non-Newtonian flows in circular and annular ducts are of particular interest in processes involving double-pipe and triple-pipe heat exchangers, whose design is not a trivial operation. This problem mainly concerns food or chemical industries, when products to be treated may exhibit complex rheological behavior, such as fruit purees, emulsions and polymeric melts, which present high apparent viscosities.
Tubular heat exchangers are one of the commonest types of heat exchangers in the food industry and the typical areas of application are pasteurization and sterilization of fruit concentrates and vegetable purees (Sannervik et al., 1996). In this way, non-dimensional correlations for heat transfer in fully developed laminar flows of pseudoplastic fluids in circular and concentric annular geometries during forced convection at the thermal entrance are essential in the modeling, optimization and design of these processes.
Many investigations have suggested a number of analytical, semi-analytical and empirical correlations for circular ducts; however, especially for the case of annular regions, whose studies are primarily devoted to numerical analyses, experimental data are of particular importance in order to confirm theoretical evaluations.
As observed by Cho and Hartnett (1982), since the early theoretical work of Graetz, in 1883 and 1885, Nusselt, in 1910, and Lévêque, in 1928, the analysis of the laminar heat transfer performance in the thermal entrance region of Newtonian fluids flowing in circular tubes has been carried out in different ways by many investigators, for various boundary conditions. Some of these results are summarized in Shah and London (1978), Shah and Bhatti (1987) and Kays and Crawford (1993). Additionally, also available in the literature is a great deal of information on the effects of viscous dissipation (Krishnan and Sastri, 1978; Lawal and Mujumdar, 1985) and fluid axial conduction (Vick and Ozisik, 1981; Bilir, 1992; Olek, 1998). In the last fifty years, many extensions of the widely known Graetz problem (or Graetz-Nusselt problem) and the Lévêque solution have been proposed; in addition, the analyses of the accuracy of analytical and numerical results have received attention. We can cite some important contributions to this field, such as Lyche and Bird (1956) who extended the Graetz-Nusselt problem to Power-Law flow and Pigford (1955), who first modified the Lévêque approach to Power-Law fluids. Subsequently, Metzner et al. (1957) used a consistency empirical radial correction in the extended Lévêque equation, as that one used by Sieder and Tate (1936) to account for radial variations in fluid consistency, while Christiansen and Craig (1962) have shown that the effects of radial viscosity variation may be treated by an analytical method, described by an exponential relationship. Popovska and Wilkinson (1977), Gori (1978), Cotta and Ozisik (1986), Mansour (1989), Prusa and Manglik (1994) and Khellaf and Lauriat (1997) also studied the heat transfer by forced convection in the thermal entrance of circular pipes for hydrodynamically developed flows of Power-Law fluids in the case of negligible viscous dissipation and axial heat conduction in the fluid, under conditions of constant surface temperatures, while Bird et al. (1977) and Cotta and Ozisik (1986) considered prescribed wall heat fluxes. Natural convection effects introduced by fluid density variations were studied by Mahalingam et al. (1975) and Basset and Welty (1975), among others, for uniformly constant heat flux at the wall, while for constant wall temperature average Nusselt correlations were proposed, for example, by Metzner and Gluck (1960), Oliver and Jenson (1964), Gori (1978) and Rodriguezluna et al. (1987). The effect of heat generation by viscous dissipation was included by Shih and Tsou (1978), Richardson (1979), Lawal and Mujumdar (1989), Ramachandran (1993), Manglik and Prusa (1995) and Barletta (1997), and convection in the region where laminar flow is fully developed both thermally and hydrodynamically was studied by Skelland (1967) and Barletta (1997), whereas the simultaneous development of the laminar flow and the convective heat transfer in short circular ducts have been experimentally analyzed by Fontes and Gasparetto (2001).
On the other hand, works on the problem of convective heat transfer in the thermal entrance region of annular ducts during flow of Power-Law fluids are rather scarce, while for Newtonian fluids an extensive literature exists both on forced convection (Lundberg et al., 1963; Moghadam and Aung, 1990; Barletta et al., 2004) and mixed convection (Nonino and Delgiudice, 1996; Barletta and Lazzari, 2005), including the effects of eccentricity (Cheng and Hwang, 1968; Trombetta, 1971; El-Shaarawi et al., 1998), viscous dissipation (Rokerya and Igbal, 1971) and inner or outer cylinder rotation (Childs and Long, 1996). For Power-Law flows, investigations are almost reduced to the case of hydrodynamic and thermal fully developed conditions, and the solutions of the problem are basically obtained through finite-difference techniques, except for the works of Tanaka and Mitsuishi (1975) and Nouar et al. (2000); the former not only analyzed numerically but also experimentally the problem of combined forced and free convection in the thermal entrance of a fully developed laminar flow in a horizontal annulus under the conditions of a constant-temperature inner wall and an insulated outer wall, whereas the latter considered the outer and inner cylinders uniformly heated with a constant heat flux density. The relatively recent studies of Manglik and Fang (2002) and Manglik and Fang (1995) considered the forced convection heat transfer in eccentric annular ducts with an insulated outer surface and uniform axial heat flux or constant wall temperature for the inner surface. Barletta (2000) investigated analytically the forced convection in a vertical annular duct with the boundary surfaces supposed to be isothermal with unequal temperatures. Batra and Sudarsan (1992) considered the case of the simultaneous development of the hydrodynamic and thermal boundary layers, in which one wall of the annulus was isothermal and the other adiabatic, while Capobianchi and Irvine (1992) examined fully developed heat transfer characteristics of a modified Power-Law fluid. Finally, we can cite Hong and Matthews (1969), who probably first solved analytically the heat transfer to Power-Law fluids in the thermal entrance region of annuli.
Recognizing the need of experimental results, extremely scarce in the literature and essential to validate theoretical analyses, the aim of this work was to obtain heat transfer coefficients, as well as average Nusselt numbers, in the laminar flow of egg yolk - a pseudoplastic fluid with important potential for the international market - through circular and annular ducts with constant surface temperatures, calculated from experimentally measured inlet and outlet bulk temperatures. The results were compared with existing correlations for Newtonian and non-Newtonian fluids and the influence of the annulus aspect ratio on the Nusselt number was considered.
MATERIALS AND METHODS
Samples: Liquid Egg Yolk
The liquid egg yolk for this study was obtained directly from the processing line of an egg breaking plant. The moisture content of the initial batch was determined by the gravimetric method using a vacuum oven (48 hrs, 333 K, 100 mmHg), resulting in 54.04(± 0.43)% of moisture (wet basis). The sample pH was 6.40 ± 0.04.
Thermophysical Properties of Liquid Egg Yolk
Specific heat, thermal conductivity, density and rheological parameters of liquid egg yolk at various temperatures were determined using the equations described by Gut et al. (2005). The calculated thermophysical properties are shown in Table 1.
All the fluid properties were estimated as the arithmetic average of inlet and outlet bulk temperatures, Tba =(Tb1 + Tb2 )/2 , in which Tb1 and Tb2 (ºC) are, respectively, the inlet and outlet bulk temperatures of the fluid.
Experimental Apparatus for Determination of the Bulk Temperatures
The experimental apparatus used for measuring average heat transfer coefficients in horizontal ducts of circular and annular cross sections was similar to the equipment used by Telis-Romero et al. (2006) when studying hydrodynamics of egg yolk flowing in a circular pipe.
Two heat transfer units were used. One of the units was made with four horizontal steel circular tubes, with nominal diameters of 1/4 in, 1/2 in, 1 in and 1½ in; the total length of this test section was 1.2 m, providing a maximum length-to-diameter ratio (L/D) of 187.5. The other unit consisted of two sections of triple-pipe heat exchangers, with different radius ratio for the inner annulus and a total length of 1.2 m. The triple-pipe heat exchanger was formed by three coaxial cylinders, in which water from a thermostatic bath flowed in the tube and the outer annulus, while the liquid egg yolk flowed in the inner annulus. The external diameter of the tube (D1) was fixed at 0.0137 m, while the internal diameter of the cylinder forming the inner annulus (D2) was 0.0274 m, 0.0381 m, 0.0590 and 0.0727 m, thus providing the respective values of 0.0137 m, 0.0244 m, 0.0453 and 0.0590 m for the hydraulic diameter (Dh), and the respective values of 0.500, 0.360, 0.059 and 0.0727 m for the annulus aspect ratio (κ). The maximum length-to-diameter ratio (L/Dh) was 87.6.
The heat transfer sections were submerged in a large thermostatic bath (Model MA-184, Marconi, SP, Brazil), in which water flowed at a high mass flow rate and at a constant temperature ( 66 C). The experiments were carried out during samples heating by using water in the thermostatic bath. A distance of 1.0 m provided the developing length of the flow regime, guaranteeing the establishment of the velocity profile at the entrance of the heating zone. Temperature transducers (Model TT-302, Smar, SP, Brazil) were used to measure temperature at the beginning and at the end of the test section. A static mixer was placed at the end of the two pieces of the equipment to homogenize the final temperature of the liquid egg yolk, according to Figure 1. Copper-constantan thermocouples were soldered into grooves along the heating test sections to measure tube wall temperature. In all the experiments, the wall temperature difference between the first and the last copper-constantan thermocouple was approximately 0.6 ºC. When readings were taken, an effort was made to keep the average temperature of the fluid constant for several values of velocity so that its effect could be isolated from that of other factors, as suggested by Sieder and Tate (1936). The egg yolk was pumped by means of a peripheral pump (Model P-500, KSB, SP, Brazil). A flow meter (Model LD100, MLW, Germany) was used to initially adjust the desired flow rate in each experiment, but exact measurements were obtained by weighing fluid samples collected at determined time intervals. A data logger running a data acquisition and control program monitored temperatures and pressures. The average flow velocities were varied from 0.14 to 2.00 m.s-1, adding up to one hundred thirty-six experimental values for the circular cross section and two hundred eighty-eight points for the annular geometry.
The performance of the apparatus was checked using a CMC solution (1% w/w), which was pumped through the equipment at 68 different flow rates in the laminar range. At each flow rate, inlet and outlet temperatures in a straight pipe section of 1.80 m were measured with ten repetitions made at five-minute intervals, in a procedure similar to that used by Polizelli et al. (2003) and Telis-Romero et al. (2005) when investigating friction losses in fittings during non-Newtonian fluid flow. The thermophysical properties of CMC solution were obtained from the work of Carezzato et al. (2007).
Calculation of the Heat Transfer Coefficients and Development of the Average Nusselt Correlation
Considering a fluid flowing through a circular tube of diameter D , in which there is a heated wall section of length L, whose inside surface temperature is T0 (z), a steady-state energy balance over a length (Bird et al., 2002) is made by stating that the heat through the walls plus the energy entering at z = 0 by convection equals the energy leaving the tube at z = L. For fully developed flows, changes in the kinetic energy flux and the work term will be negligible relative to changes in the enthalpy flux. Then, by neglecting the axial heat conduction term, the steady-sate energy balance becomes simply "rate of energy flow in = rate of energy flow out". Combining the energy balance equation with the conventional definition for the heat flow into the fluid, one may obtain Eq. (1).
in which hIn (W.m-2.ºC-1) is the heat transfer coefficient based on the corresponding logarithmic mean temperature difference ΔTln (ºC), (kg.s-1) is the total mass flow rate, Cp is the fluid specific heat (J.kg-1.ºC-1), Tb1 and Tb2 (ºC) are respectively the inlet and outlet bulk temperatures of the fluid, D (m) is the tube internal diameter, L (m) is the tube length and T0 (ºC) is the tube wall temperature. Therefore, the Nusselt numbers may be obtained from Eq. (2).
in which k is the thermal conductivity of the fluid (W.m-1.ºC-1). The dimensionless numbers Gz , Remr and Prg, given respectively by Eqs. (3), (4) and (5), may be used to correlate new empirical expressions for the Nusselt number.
in which ρ is the fluid density (kg.m-3) and is the average axial flow velocity (m.s-1).
All fitted functions were performed using Nonlinear Estimation Procedures from the software MatLab 6.0® (The MathWorks Inc., 2001) which solves nonlinear curve fitting problems in the least square sense. The adequacy of the fitted functions was evaluated by the correlation coefficient (R2), the magnitude of the root mean square (RMS) error calculated according to Gabas et al. (2002), the chi-square values of fit (Chi2), the sum of squares of the difference between data and fit values (SSR), and the absolute relative errors between observed and predicted values, as given by Telis-Romero et al. (2001).
RESULTS AND DISCUSSION
Heat Transfer in Circular Ducts
The heating experiments in circular sections permitted the evaluation of hln according to Eq. (1). Nusselt and Graetz numbers were obtained by Eqs. (2) and (3), respectively.
The experimental conditions can be summarized as follows:
■ The flow was steady (the flows were sustained for ten minutes before the temperature readings were taken), laminar, which was guaranteed by the low fluid axial velocity ( 0.14 <
<2.00 and 2.0 < Remr< 271.3 ) and fully established;■ The temperature profiles were in development; according to Prusa and Manglik (1994), the dimensionless thermal-entry length (zte*) for a Power-Law fluid with n0.333 is approximately zte* 4 × 10-1 , where zte*= z RPe and Pe is the>
■ Heat conduction in the axial direction was negligible, which is justified when Re Pr >> 100 for Newtonian fluids (Kays and Crawford 1993). The experimental conditions for the Péclet number were: 0.7 ×104 < Remr Prg< 61.7 ×104 ;
■ Heat produced by viscous dissipation could be neglected based on the Brinkman number ( Br << 1); the experimental conditions were: 4.2 ×10-4< Br < 5.4 ×10-2 ;
■ Thermophysical properties of the fluid were almost constant between the tube entrance and the exit for each experimental test. The maximum variation of density in an experimental test was 8.3 kg.m-3, with a mean variation for all the experiments of 1.8 kg.m-3, while the maximum and mean variations of specific heat were 62.3 kJ.kg-1.ºC-1 and 11.8 kJ.kg-1.ºC-1, respectively. For thermal conductivity, the maximum variation in an experimental test was 0.014 W.m-1.ºC-1, and the mean variation for all experiments was 0.004 W.m-1.ºC-1;
■ Natural convection effects could be neglected, which is justified by the nearly constant values of density between the tube inlet and outlet for all experimental tests;
■ The flow behavior index presented a mean value for all the experiments of 0.855 and a standard deviation of 0.004, while the consistency index ranged from 0.10 to 2.3 Pa sn;
■ The ranges tested were: 28.5 < Gz < 14457.6 and 31.3 < LD < 184.9 .
Based on the conditions attained in the experiments, the correlation proposed by Metzner et al. (1957), given by Eq. (6), was compared with the experimental results. Metzner et al. (1957) presented the first theoretical analysis combined with an experimental study of the variables controlling heat transfer rates to non-Newtonian fluids; their experimental data covered the ranges: 0.3×104 < Gz < 0.01×104 and 0.18 < n < 0.70 . Their expression for predicting average Nusselt numbers was obtained for a constant property, hydrodynamically fully developed, thermally developing, laminar flow through a circular tube, with negligible axial conduction and viscous dissipation. The tested fluids were aqueous CMC (carboxymethylcellulose) solutions and aqueous Carbopol solutions.
in which:
The Lévêque equation describing heat transfer to a Newtonian fluid was also compared with the experimental data, as well as other correlations for non-Newtonian fluids, such as those proposed by Pigford (1955), Gori (1978), Joshi and Bergles (1982) and Prusa and Manglik (1994). As observed by Metzner et al. (1957) and Christiansen and Craig (1962), predictions from the Lévêque equation deviate from the non-Newtonian behavior. As would be expected, the data lie considerably above the theoretical Newtonian curve with a RMS error value δ1/3 of 21.6 %. Introduction of the factor brings the data more nearly in line with the theoretical curve predicted by Pigford's expression, but there is still some scatter (RMS error = 12.1 %). The empirical correction factor (K/ K0)0.14 to account for the distortion of the theoretical velocity profile adequately correlates the experimental results; in that case, the root mean square error was the smallest of all (RMS error = 8.9 %). Obviously, the presence of some experimental error was indicated in the work of Metzner et al. (1957), which points out some inaccuracy in the curve predicted by Eq (6). Also, in the present work there is some imprecision in the Nusselt predictions, since all fluid properties were estimated from empirical equations, which present some inherent deviations. Although some authors such as Christiansen and Craig (1962), Popovska and Wilkinson (1977) and Forrest and Forrest and Wilkilson (1973) had doubts about the accuracy of Eq. (6), all deviations are within experimental errors, and for design purposes Eq. (6) is perfectly acceptable. The expressions of Gori (1978), Joshi and Bergles (1982) and Prusa and Manglik (1994) provided similar results among themselves, with RMS error 15% , but evidently Eq. (6) displayed the best predictions.
In order to evaluate the performance of the system, experimental data obtained during flow of a CMC solution (1%, w/w) through the circular duct were used. Pipe dimensions, thermophysical properties and measured temperatures were substituted into Equations (2) and (3) to give the Graetz and Nusselt numbers, which were then correlated by Equation (6). These results are shown in Figure 2. The agreement between experimental and predicted values was very satisfactory, indicating the adequacy of the equipment and methodology used.
Eq. (6) has been widely used for engineering design purposes, and the good agreement between observed data and its predictions is clearly evident in Fig. 3 for liquid egg yolk. The experimental results obtained in this work were also submitted to nonlinear regression analysis, resulting in Eq. (8) with R2 = 0.926 and SSR = 2569. Eq. (9), similar to Eq. (6), was also fitted to the experimental data and resulted in R2 = 0.921 and SSR = 1098.
Heat Transfer in Annular Ducts
The heating experiments in the annular regions permitted evaluation of hln , Nusselt and Graetz according to Eqs. (1), (2) and (3), respectively, using the concept of the hydraulic diameter. The experimental conditions can be summarized as follows:
■ The flow was steady, laminar ( 0.14 <
< 2.00 and 42.8 < Remr< 2263.4 ) and fully established;■ Conduction of heat in the axial direction was negligible ( Pe >> 100 ); the experimental conditions for the Péclet number were: 2.1×104 < Remr Prg< 4.5 ×105 ;
■ Heat produced by viscous dissipation could be neglected ( Br << 1); the experimental conditions were: 1.6 ×10-3< Br < 7.8 ×10-2 ;
■ The thermophysical properties of the fluid were almost constant between entrance and exit of the test section for each experimental test. The maximum variation between inlet and outlet bulk temperatures was 6.9ºC, with a mean variation of 2.8º for all experimental tests, which indicated small fluctuations of thermal properties;
■ Natural convection effects could be neglected, which is justified by the nearly constant values of density between tube inlet and outlet for all experimental tests;
■ The flow behavior index presented a mean value for all experiments of 0.855 and a standard deviation of 0.004, while the consistency index ranged from 0.10 to 2.3 Pa sn;
■ The ranges tested were: 130.6 < Gz < 34000 , 20.3 < L/Dh< 87.6 and 0.188 < κ (D1/D2) < 0.50 .
An equation similar to that proposed by Metzner et al. (1957) for pseudoplastic fluids flowing in circular ducts (Eq. 6) was tested using the hydraulic diameter (Dh) instead of the pipe diameter D. The adjustment resulted in Eq. (10), but in spite of the R2 value of 0.949, experimental data lay systematically above the prediction curve.
Still based on Metzner's correlation, another expression was proposed, in which an empirical factor was added to account for the effect of the radius ratio κ (D1/D2) of the annulus. The final proposed correlation for arbitrary values of κ ranging from 0.188 to 0.50 is given by Eq. (6), and was based on the fitting models given by Levenspield (1993) for flow of Newtonian fluids through an annulus. The statistical results were quite satisfactory: R2 = 0.960 and SSR = 8142. The experimental results, along with predictions of Eq. (11), are presented in Fig. 4.
Little effort has been undertaken in collecting experimental data on heat transfer to Newtonian fluids in annuli for the laminar and turbulent regions. Some correlations can be found in Jakob (1949), which are generally given in terms of the aspect annulus ratio and the Graetz number, or the Reynolds and Prandtl numbers. To the authors' knowledge, just one correlation exists to determine the mean convective heat transfer coefficient for Power Law fluids flowing in concentric annular ducts, and very little experimental data are available. Tanaka and Mitsuishi (1975) obtained some experimental results for aqueous CMC solutions, aqueous PEO (polyethylene oxide) solutions and aqueous SPA (sodium polyacrylate) solutions flowing through annuli with κ = 0.354 and 0.746. They noticed that, since the Power Law fluid is generally highly viscous, the value of Gr Re is small. Based on their numerical results, they proposed an expression for the Nusselt number, which was a function of κ, n, Re, Gz and Gr; their experimental results and the proposed equation agree within 15 %. In the experimental tests of Nouar et al. (2000) with CMC aqueous solutions, the variation range of the working conditions was: 200 < Pr <1520 , 18 < Re <131 and 233 < Gr <22,350 . The two cylinders of the test cell were heated by passing an electrical current through the tube wall, and their test section was covered by a thick polyurethane layer. Unfortunately, they did not express their results in terms of the average Nusselt number, and also did not provide a simple relationship for estimating it. They were focused on the effect of the variation of the rheological properties and that of the buoyancy force on the flow structure. They found that, in the case of forced convection coupled with a consistency variation with temperature, the flow structure is characterized by a displacement of fluid particles from the core region towards the heated wall. Then, the wall velocity gradient increases and the axial velocity in the central zone of the annular gap decreases to maintain the flow rate conservation; the deformation rate of the axial velocity profile decreases along the heating zone. As a matter of fact, in the boundary layer, the flow is quickly accelerated from the entrance, then the acceleration declines downstream. Therefore, they concluded that forced convection is the dominant mechanism near the entrance and the core flow is decelerated because of the decrease of the consistency index K as the temperature increases near the heated walls. In addition, they showed that the thermo-dependency effect of K is more characterized with increased shear-thinning of the fluid.
CONCLUSIONS
Heat transfer to laminar, hydrodynamically fully developed flow of a pseudoplastic fluid - egg yolk - in the thermal entrance of circular and annular ducts with constant wall temperatures had been experimentally investigated. The average convection heat transfer coefficients, as well as the average Nusselt numbers, were obtained by measuring inlet and outlet temperatures during heating of liquid egg yolk in the ducts test sections. For circular ducts, the experimental data were well correlated by empirical equations (Eqs. 8 and 9) and by the classic solution proposed by Metzner et al. (1957) for the thermal-entry and fully developed laminar flow of Power Law fluids. For annular regions, a simple empirical expression was developed based on the work of Metzner et al. (1957) and by adding an empirical factor to account for the effect of the radius ratio κ (D1/D2) of the annulus (Eq. 11). The new correlation, developed for design purposes of heat transfer devices, such as the triple-pipe or double-pipe heat exchangers, was obtained in the following ranges: 130.6 < Gz < 34000 , 42.8 < Remr< 2263.4 , 20.3 < LD < 87.6 , 0.188 < κ (D1/D2) < 0.50 , 0.10 < K < 2.3 and n = 0.855 ±0.006 .
NOMENCLATURE
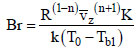



ACKNOWLEDGMENTS
The authors would like to thank the National Council for Scientific and Technological Development, CNPq, and Sao Paulo State Research Fund Agency, FAPESP (Proc. 2002/02461-0), for their financial support.
(Submitted: August 6, 2007 ; Revised: November 21, 2007 ; Accepted: December 27, 2007)
- Barletta, A. Combined forced and free flow of a power-law fluid in a vertical annular duct, International Journal of Heat and Mass Transfer, 43, No. 19, 3673 (2000).
- Barletta, A. Fully developed laminar forced convection in circular ducts for power-law fluids with viscous dissipation, International Journal of Heat and Mass Transfer, 40, No. 1, 15 (1997).
- Barletta, A., Lazzari, S. Forced and free flow in a vertical annular duct under nonaxisymmetric conditions, Journal of Heat Transfer-Transactions of the ASME, 127, No. 6, 606 (2005).
- Barletta, A., Lazzari, S. Zanchini, E. Convection in a vertical annular duct with circumferentially variable boundary conditions, Journal of Thermophysics and Heat Transfer, 18, No. 4, 563 (2004).
- Bassett, C. E., Welty, J. R. Non-Newtonian heat-transfer in thermal entrance region of uniformly heated, horizontal pipes, Aiche Journal, 21, No. 4, 699 (1975).
- Batra, R. L., Sudarsan, V. R. Laminar-flow heat-transfer in the entrance region of concentric annuli for power law fluids, Computer Methods In Applied Mechanics and Engineering, 95, No. 1, 1 (1992).
- Bilir, S. Numerical-solution of graetz problem with axial conduction, Numerical Heat Transfer Part A-Applications, 21, No. 4, 493 (1992).
- Bird, R. B., Armstrong, R. C., Hassager, O. Dynamics of polymeric liquids, John Wiley & Sons, New York (1977).
- Bird, R. B., Stewart, W. E., Lightfoot, E. N. Transport Phenomena, Second Ed., John Wiley & Sons, New York (2002).
- Carezzato, A., Alcantara, M. R., Telis-Romero, J., Tadini, C. C., Gut, J. A. W. Non-Newtonian heat transfer on a plate heat exchanger with generalized configurations, Chemical Engineering Technology, 30, No. 1, 21 (2007).
- Capobianchi, M., Irvine, T. F. Predictions of pressure-drop and heat-transfer in concentric annular ducts with modified power law fluids, Warme Und Stoffubertragung-Thermo And Fluid Dynamics, 27, No. 4, 209 (1992).
- Cheng, K. C., Hwang, G. J. Laminar forced convection in eccentric annuli, Aiche Journal, 14, No. 3, 510 (1968).
- Childs, P. R. N., Long, C. A. A review of forced convective heat transfer in stationary and rotating annuli, Proceedings of the Institution of Mechanical Engineers Part C-Journal of Mechanical Engineering Science, 210, No. 2, 123 (1996).
- Cho, Y. I., Hartnett, J. P. Non-newtonian fluids in circular pipe flow. Advances in heat transfer. Academic Press, p. 59 (1982).
- Christiansen, E. B., Craig, S. E. Heat transfer to pseudoplastic fluids in laminar flow, Aiche Journal, 8, No. 2, 154 (1962).
- Cotta, R. M., Ozisik, M. N. Laminar forced-convection of power-law non-Newtonian fluids inside ducts, Warme Und Stoffubertragung-Thermo and Fluid Dynamics, 20, No. 3, 211 (1986).
- Cotta, R. M., Ozisik, M. N. Laminar forced-convection to non-Newtonian fluids in ducts with prescribed wall heat-flux, International Communications in Heat and Mass Transfer, 13, No. 3, 325 (1986).
- El-Shaarawi, M. A. I., Abualhamayel, H. I., Mokheimer, E. M. A. Developing laminar forced convection in eccentric annuli, Heat and Mass Transfer, 33, No. 5-6, 353 (1998).
- Fontes, S. R., Gasparetto, C. A. Convective laminar flow of food additives solutions in circular entrance region, International Communications in Heat and Mass Transfer, 25, No. 5, 693 (2001).
- Forrest, G., Wilkinson, W. L. Laminar heat transfer to power law fluids in tubes with constant wall temperature, Transactions of the Institute of Chemical Engineers, 51, 331 (1973).
- Gabas, A. L., Menegalli, F. C., Ferrari, F., Telis-Romero, J. Influence of drying conditions on the rheological properties of prunes, Drying Technology, 20, No. 7, 1485 (2002).
- Gori, F. Effects of variable physical-properties in laminar-flow of pseudoplastic fluids, International Journal of Heat and Mass Transfer, 21, No. 2, 247 (1978).
- Gut, J.A.W., Pinto, J.M., Gabas, A.L., Telis-Romero, J. Continuous pasteurization of egg yolk: thermophysical properties and process simulation. Journal of Food Process Engineering, 28, 181 (2005).
- Hong, S. N., Matthews, J. C. Heat transfer to non-Newtonian fluids in laminar flow through concentric annuli, International Journal of Heat and Mass Transfer, 12, No. 12, 1699 (1969).
- Jakob, M. Heat transfer, John Wiley & Sons, New York (1949).
- Joshi, S. D., Bergles, A. E. Heat transfer to laminar flow of non-Newtonian pseudoplastic fluids in tubes, Heat transfer, Hemisphere, Washington, DC, p. 51 (1982).
- Kays, W. M. M., Crawford, E. Convective heat and mass transfer, Third ed., McGrawHill, New York (1993).
- Khellaf, K., Lauriat, G. A new analytical solution for heat transfer in the entrance region of ducts: Hydrodynamically developed flows of power-law fluids with constant wall temperature, International Journal of Heat and Mass Transfer, 40, No. 14, 3443 (1997).
- Krishnan, K. N., Sastri, V. M. K. Numerical-solution of thermal entry length problem with variable viscosities and viscous dissipation, Warme Und Stoffubertragung-Thermo And Fluid Dynamics, 11, No. 2, 73 (1978).
- Lawal, A., Mujumdar, A. S. Extended Graetz problem -a comparison of various solution techniques, Chemical Engineering Communications, 39, No. 1-6, 91 (1985).
- Lawal, A., Mujumdar, A. S. Viscous dissipation effects on thermal entrance heat-transfer to power law fluids in arbitrary cross-sectional ducts, Chemical Engineering Journal and the Biochemical Engineering Journal, 41, No. 2, 57 (1989).
- Levenspield, O. Engineering Flow and Heat Exchange, Plenum Press, New York, 1993.
- Lundberg, R. E., Mccuen, P. A., Reynolds, W. C. Heat transfer in annular passages. Hydrodynamically developed laminar flow with arbitrarily prescribed wall temperatures or heat fluxes, International Journal of Heat and Mass Transfer, 6, 495 (1963).
- Lyche, B. C., Bird, R. B. The Graetz-Nusselt problem for a power-law non-Newtonian fluid, Chemical Engineering Science, 6, No. 1, 35 (1956).
- Mahalingam, R., Tilton, L. O., Coulson, J. M. Heat-transfer in laminar-flow of non-Newtonian fluids, Chemical Engineering Science, 30, No. 8, 921 (1975).
- Manglik, R. M., Fang, P. P. Effect of eccentricity and thermal-boundary conditions on laminar fully-developed flow in annular ducts, International Journal of Heat and Fluid Flow, 16, No. 4, 298 (1995).
- Manglik, R. M., Fang, P. Thermal processing of viscous non-Newtonian fluids in annular ducts: Effects of power-law rheology, duct eccentricity, and thermal boundary conditions, International Journal of Heat and Mass Transfer, 45, No. 4, 803 (2002).
- Manglik, R. M., Prusa, J. Viscous dissipation in non-Newtonian flows - implications for the nusselt number, Journal of Thermophysics and Heat Transfer, 9, No. 4, 733 (1995).
- Mansour, A. R. An analytical solution of laminar heat-transfer to power-law non-Newtonian fluids in circular ducts Graetz-Nusselt problem, International Communications in Heat and Mass Transfer, 16, No. 2, 199 (1989).
- Matlab. 2001, The MathWorks Inc.: USA.
- Metzner, A. B., Gluck, D. F. Heat transfer to non-Newtonian fluids under laminar flow conditions, Chemical Engineering Science, 12, No. 3, 185 (1960).
- Metzner, A. B., Vaughn, R. D., Houghton, G. L. Heat transfer to non-Newtonian fluids, Aiche Journal, 3, No. 1, 92 (1957).
- Moghadam, H., Aung, W. Numerical method for laminar convection in a concentric vertical annular duct with variable properties, Numerical Heat Transfer, 18A, 357 (1990).
- Nonino, C., Delgiudice, S. Finite element analysis of laminar mixed convection in the entrance region of horizontal annular ducts, Numerical Heat Transfer Part A-Applications, 29, No. 3, 313 (1996).
- Nouar, C., Benaouda-Zouaoui, B., Desaubry, C. Laminar mixed convection in a horizontal annular duct. Case of thermodependent non-Newtonian fluid, European Journal of Mechanics B-Fluids, 19, No. 3, 423 (2000).
- Olek, S. Heat transfer in duct flow of non-Newtonian fluids with axial conduction, International Communications in Heat and Mass Transfer, 25, No. 7, 929 (1998).
- Oliver, D. R., Jenson, V. G. Heat transfer to pseudoplastic fluids in laminar flow in horizontal tubes, Chemical Engineering Science, 19, No. 2, 115 (1964).
- Pigford, R. L. Nonisothermal flow and heat transfer inside vertical tubes, Chemical Engineering Progress Symposium Series, 51, No. 17, 79 (1955).
- Polizelli, M. A., Menegalli, F. C., Telis, V. R. N., Telis-Romero, J. Friction Losses in Valves and Fittings for Power-Law Fluids. Brazilian Journal of Chemical Engineering, 20, No. 04, 455 (2003).
- Popovska, F. W., Wilkinson, L. Laminar heat-transfer to Newtonian and non-Newtonian fluids in tubes, Chemical Engineering Science, 32, No. 10, 1155 (1977).
- Prusa, J., Manglik, R. M. Asymptotic and numerical-solutions for thermally developing flows of Newtonian and non-Newtonian fluids in circular tubes with uniform wall temperature, Numerical Heat Transfer Part a-Applications, 26, No. 2, 199 (1994).
- Ramachandran, P. A. Boundary integral solution method for the Graetz problem, Numerical Heat Transfer Part B-Fundamentals, 23, No. 2, 257 (1993).
- Richardson, S. M. Extended leveque solutions for flows of power law fluids in pipes and channels, International Journal of Heat and Mass Transfer, 22, No. 10, 1417 (1979).
- Rodriguezluna, G., Segurajauregui, J. S., Torres, J., Brito, E. Heat-transfer to non-Newtonian fluid foods under laminar-flow conditions in horizontal tubes, Journal of Food Science, 52, No. 4, 975 (1987).
- Rokerya, M. S., Iqbal, M. Effects of viscous dissipation on combined free and forced convection through vertical concentric annuli, International Journal of Heat and Mass Transfer, 14, No. 3, 491 (1971).
- Sannervik, J., Bolmstedt, U., Tragardh, C. Heat transfer in tubular heat exchangers for particulate containing liquid foods, Journal of Food Engineering, 29, No. 1, 63 (1996).
- Shah, R. K., Bhatti, M. S. Laminar convective heat transfer in ducts, in: S. Kakaç, R. K. Shah, W. Aung (Eds.), Handbook of single-phase convective heat transfer, John Wiley & Sons, New York, p. 3.1-3.137 (1987).
- Shah, R. K., London, A. L. Laminar flow forced convection in ducts, Academic Press, New York (1978)
- Shih, Y. P., Tsou, J. D. Extended leveque solutions for heat-transfer to power law fluids in laminar-flow in a pipe, Chemical Engineering Journal and the Biochemical Engineering Journal, 15, No. 1, 55 (1978).
- Sieder, E. N., Tate, G. E. Heat transfer and pressure drop of liquids in tubes, Industrial and Engineering Chemistry, 28, 1429 (1936).
- Skelland, A. H. Asymptotic rates of heat or mass transfer in non-Newtonian laminar flow, Industrial & Engineering Chemistry Fundamentals, 6, No. 1, 148 (1967).
- Tanaka, M., Mitsuishi, N. Non-Newtonian laminar heat transfer in concentric annuli, Heat Transfer Japanese Research, 4, 26 (1975).
- Telis-Romero, J., Cabral, R. A. F., Gabas, A. L., Telis, V. R. N. Rheological properties and fluid dynamics of coffee extract, Journal of Food Process Engineering, 24, No. 4, 217 (2001).
- Telis-Romero, J. T., Polizelli, M. A., Gabas, A. L., Telis, V. R. N. Friction Losses in Valves and Fittings for Viscoplastics Fluids. Canadian Journal of Chemical Engineering, v.83, p.181 (2005).
- Telis-Romero, J., Thomaz, C. E. P., Bernardi, M., Telis, V. R. N., Gabas, A. L. Rheological properties and fluid dynamics of egg yolk, Journal of Food Engineering, 74, 191 (2006).
- Trombetta, M. L. Laminar forced convection in eccentric annuli, International Journal of Heat and Mass Transfer, 14, No. 8, 1161 (1971).
- Vick, B., Ozisik, M. N. Effects of axial conduction and convective boundary-conditions in slug flow inside a circular tube, Journal of Heat Transfer-Transactions of the Asme, 103, No. 3, 436 (1981).
Publication Dates
-
Publication in this collection
23 June 2009 -
Date of issue
June 2009
History
-
Received
06 Aug 2007 -
Reviewed
21 Nov 2007 -
Accepted
27 Dec 2007